Renewable energy sources: current state of knowledge
In addition to hydroelectricity, which accounts for over 99% of Hydro-Québec’s output, there are other renewable energy options that are in development or already well established and that have good potential.
What is hydrokinetic power?
Hydrokinetic power is the electricity generated by harnessing the kinetic energy of tides and ocean or river currents using a turbine. Hydrokinetic turbines transform the water’s energy into mechanical energy, just like wind turbines transform the wind’s energy. That energy is then converted into electricity.
There are three main types of hydrokinetic turbines:
- Vertical-axis hydrokinetic turbines
- Horizontal-axis hydrokinetic turbines
- Oscillating-foil hydrokinetic turbines
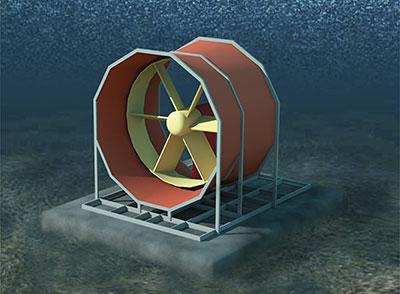
To learn more about hydrokinetic power, see the data sheet [PDF 752 Kb]
What is osmotic power?
Osmotic power is the energy derived from the difference in salinity between seawater and fresh water, which is harnessed using a turbine to generate electricity.
When fresh water is separated from seawater by a semipermeable membrane, the fresh water moves by osmosis through the membrane into the seawater, raising the pressure on the seawater side. This osmotic pressure, combined with the permeation flow rate, turns a hydraulic turbine, generating electricity.
To learn more about osmotic power, see the data sheet [PDF 1.2 Mb]
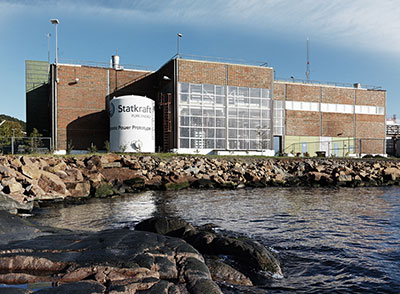
What is photovoltaic solar power?
Photovoltaic solar power is energy from sunlight, collected and converted directly into electricity by photovoltaic solar panels, also called “modules.”
The sunlight is converted into electricity in photovoltaic solar cells, using the photovoltaic effect. In a photovoltaic system, the array of cells is arranged in panels that are connected in series, in parallel or by both methods.
There are a wide range of photovoltaic technologies, all at different stages of development. Many other techniques are used to generate solar power too.
To learn more about solar power, see the data sheet [PDF 1 Mb]
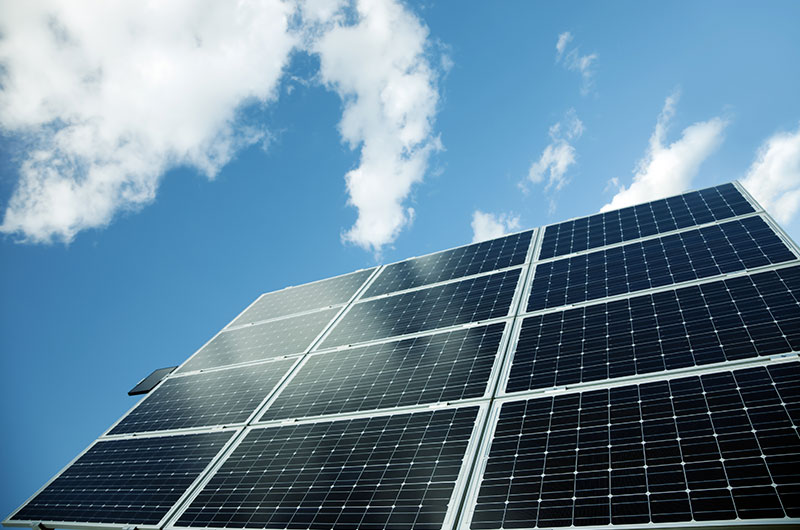
Are you interested in installing solar panels?
See our Web site to learn more about PV solar power before you invest.
What is biomass power?
Biomass power is the energy derived from animal or vegetable matter that can be converted into electricity by various methods.
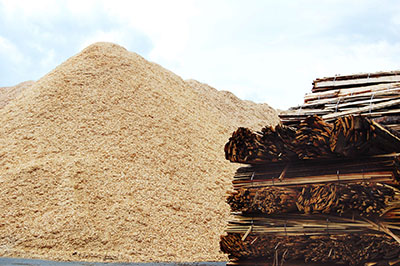
To learn more about biomass power, see the data sheet [PDF 720 Kb]
What is small wind power?
Small wind power is the kinetic energy of the wind converted into electricity by small wind turbines.
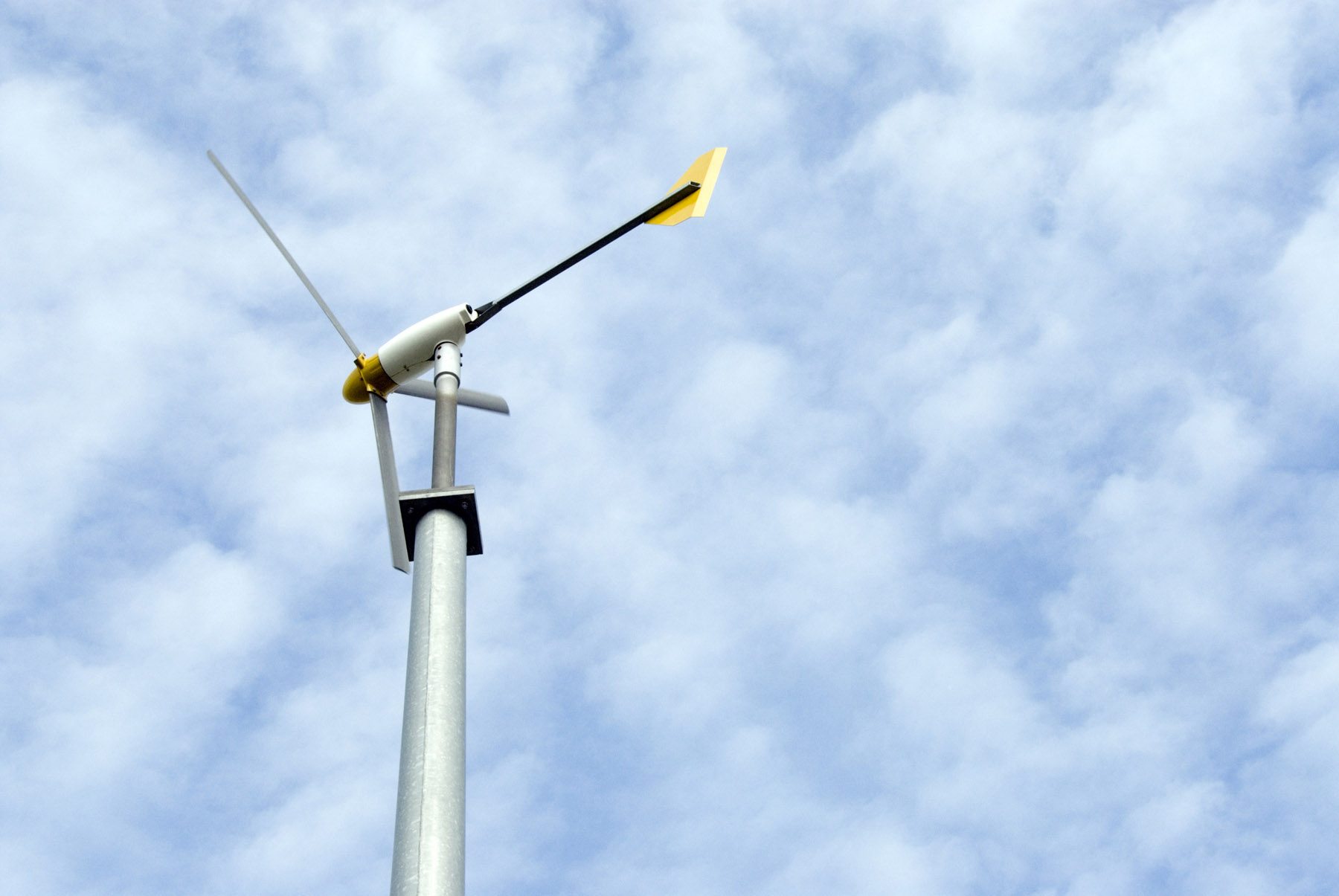
To learn more about small wind power, see the data sheet [PDF 1.3 Mb]
What is geothermal energy?
It is energy in the heat recovered from water that occurs naturally in, or has been injected into, a geothermal reservoir thousands of metres below the Earth’s surface.
Deep geothermal energy should not be confused with surface geothermal energy:
- Deep geothermal energy involves recovering heat from great depths, down to around 5,000 m, for direct heating purposes, for electric power generation using a turbine, or for both through cogeneration. The heat is extracted from the rock layers deep in the Earth’s crust using a geothermal system.
- Surface geothermal energy involves harnessing heat from the shallow layers of the Earth’s surface, down to about 400 m, for heating and cooling buildings. A heat pump is needed to convey the heat and render it compatible with the building’s heating circuit.
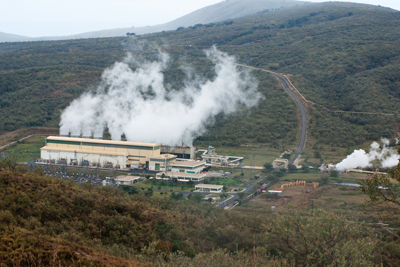
To learn more about deep geothermal energy, see the data sheet [PDF 879 Kb]
What is hydrogen?
Hydrogen (H) is the most common element in the universe. It is the main material in stars and gaseous planets. However, hydrogen is rarely found in pure form on Earth. It is most often combined with other atoms, such as oxygen, in the form of water (H2O), or carbon, in the form of hydrocarbons (CxHy). Hydrogen exists in the following two states:
- Gaseous: Two hydrogen atoms are bonded together in a form referred to as “dihydrogen” (H2).
- Liquid: Hydrogen becomes a liquid at −252.87°C.
To obtain hydrogen, it therefore has to be extracted from the molecules in which it is found. There are several methods for extracting hydrogen, including water electrolysis and steam reforming of natural gas. Producing hydrogen through water electrolysis is attractive from an environmental perspective because it does not release any carbon dioxide (CO2) and can be done locally.
Hydrogen is not a form of energy. Rather, it is an energy vector, meaning that it can carry energy obtained from a primary source for later use. Hydrogen is one of the solutions to energy storage limitations and the intermittent nature of renewable energy generation.
To date, hydrogen has been used primarily in the chemical and refining industries, but it has other potential applications in areas such as energy storage and transportation fuel.
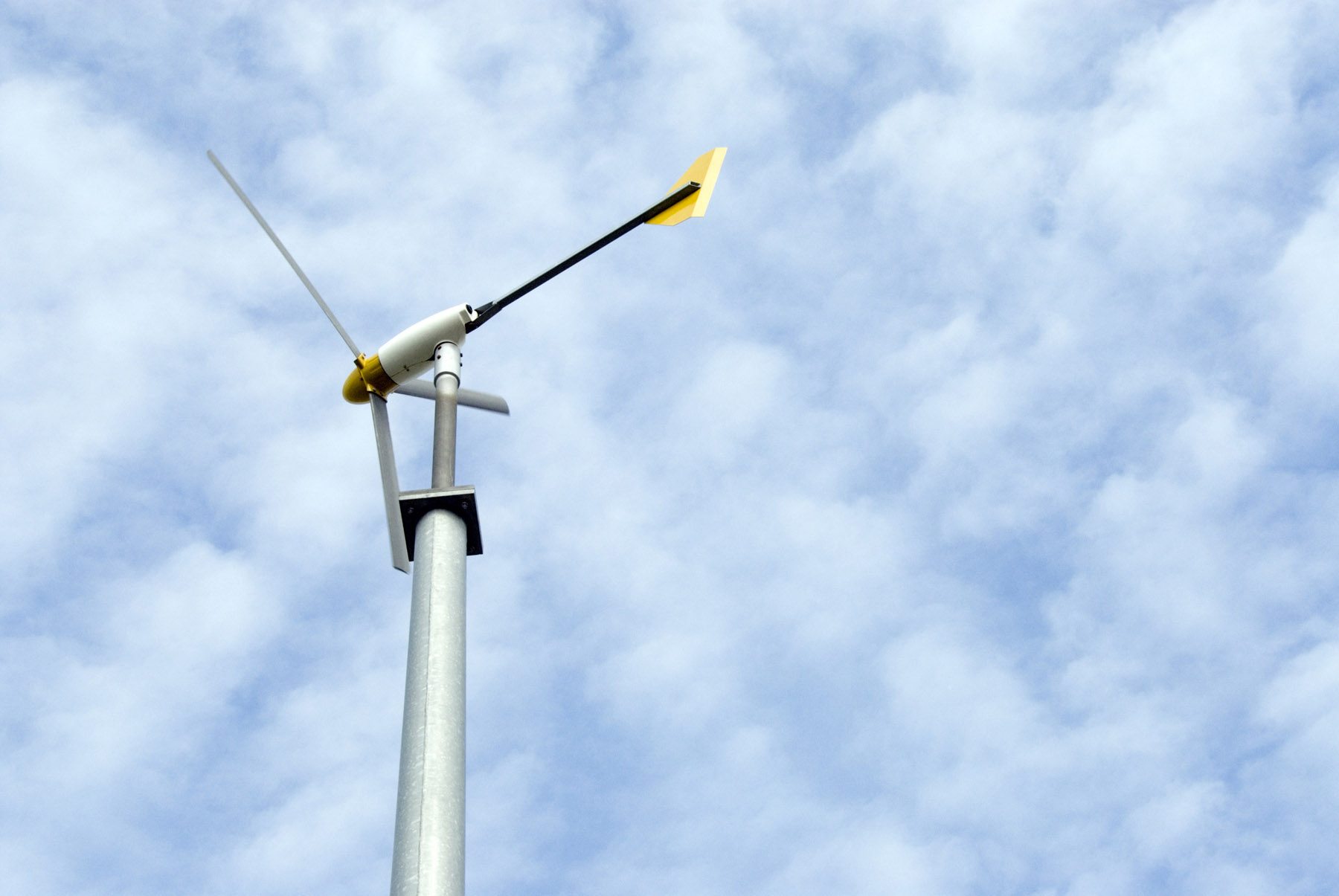
To learn more about hydrogen’s energy, see the data sheet [PDF 1.3 Mb]
- Sustainable Development Plan
- Biodiversity strategy and initiatives
- Summaries of environmental knowledge
- Net reservoir evaporation
- Greenhouse gas emissions and reservoirs
- GHG emissions and Hydro-Québec electricity
- Hydro-Québec and the mercury issue
- Hydroelectric developments and fish
- Life cycle assessment at Hydro-Québec
- Environmental follow-up
- Renewable energy sources: current state of knowledge
- Climate Change Adaptation Plan
- Hydropower Sustainability Standard certification